Lithium batteries are composed of positive electrode, negative electrode, electrolyte and separator. It has the advantages of high energy density, long cycle life, low self-discharge rate and light weight, so it is widely used in mobile equipment, electric vehicles, energy storage systems and other fields.
Classification and effects of lithium battery aging causes
1. Classification of causes of aging of lithium batteries
Lithium-ion battery aging is a complex process affected by many factors, including battery assembly method, ambient temperature, charge and discharge rate, and discharge depth.
Capacity and performance degradation are often the result of a combination of side reactions involving multiple physical and chemical mechanisms.
During the actual aging process of lithium-ion batteries, different side reactions or phase change processes will occur in different components, and these processes have different effects on capacity decline.
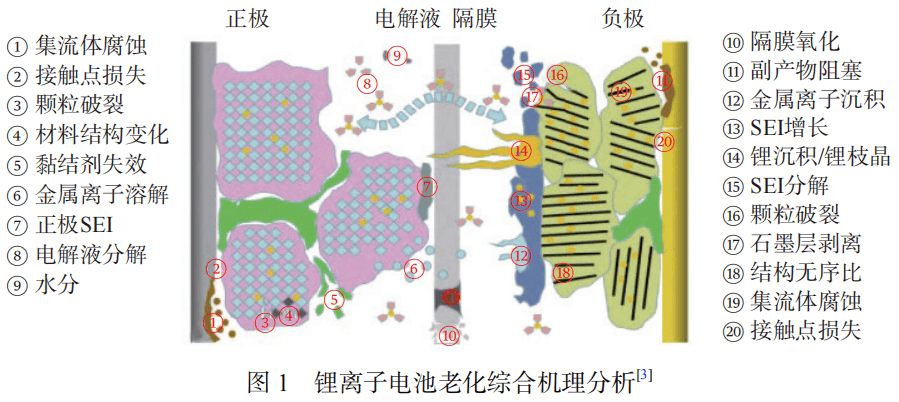
The main reasons for the capacity decline of lithium-ion batteries include SEI film growth, electrolyte decomposition, self-discharge, electrode active material loss and current collector corrosion. These factors interact during the aging process of lithium-ion batteries, causing capacity degradation.
The challenge in studying the aging mechanism of lithium-ion batteries lies in the complex coupling of various side reactions.
2. Effect of aging of lithium batteries
The aging of lithium-ion batteries will lead to problems such as reduced charge and discharge performance, usable capacity fading, and thermal stability. The decline in charge and discharge performance is manifested in extended charging time and reduced discharge capacity, which limits the battery’s usage time and endurance.
The decay of available capacity means that the energy that the battery can release is reduced, which affects the service life of the battery. Decreased thermal stability will increase the internal resistance of the battery, resulting in increased heat generation, which may lead to safety issues such as temperature rise and thermal runaway.
In addition, aging will increase the inconsistency between cells within the lithium-ion battery pack, affecting the overall performance and life of the battery pack.
Lithium battery capacity decline mechanism
1-Analysis of the impact of capacity decline caused by lithium precipitation
Lithium precipitation from the negative electrode is an important cause of aging in lithium-ion batteries. It refers to the process of lithium ions depositing from the electrolyte to the surface of the negative electrode.
It causes the loss of active lithium ions, reducing the battery’s usable capacity and performance. Controlling and reducing lithium precipitation in the negative electrode is crucial to improving battery life and safety.
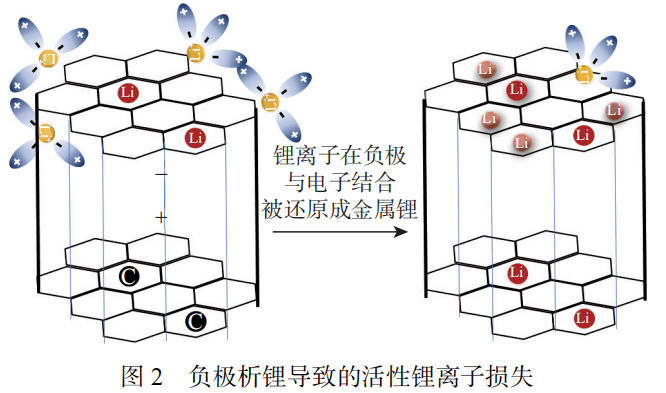
Lithium precipitation causes irreversible loss of lithium ion stock and reduces the available capacity of the battery. The growth of lithium dendrites is the main cause of the loss of active lithium ions. As shown in Figure 3, there are many factors that affect lithium deposition in batteries.
Some researchers believe that lithium evolution may occur if the rate of lithium ions embedded in the graphite negative electrode is too slow or the rate of transfer to the negative electrode is too fast.
In addition, when working under low temperature conditions, the diffusion rate of lithium ions slows down, and the working potential of the negative electrode is very close to the lithium evolution potential, so lithium evolution is more likely to occur. Factors such as too small N/P value, local electrode polarization, and geometric mismatch may also lead to the occurrence of lithium evolution.
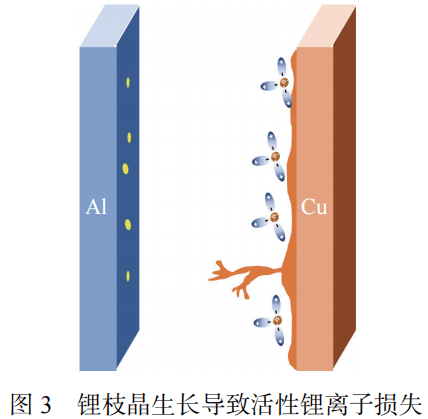
Lithium precipitation is closely related to battery aging. When there are defects inside the battery, lithium precipitation in the electrode is more likely to occur. As the battery ages, the lithium precipitation phenomenon accelerates, becoming one of the main reasons for the decline in battery capacity. Lithium precipitation will lead to a decrease in the porosity of the negative electrode and an increase in the electrolyte potential gradient, thereby accelerating battery aging. At the same time, lithium deposition leads to the loss of active lithium ions, affecting battery performance, as shown in Figure 4.
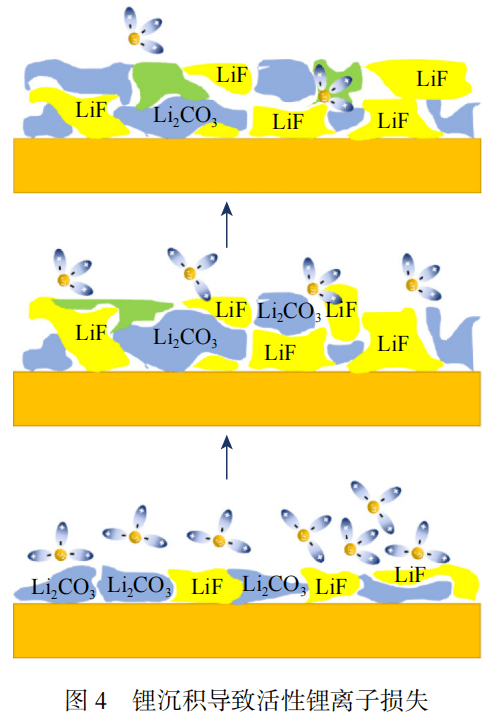
2. The impact of SEI film growth on capacity decline
The SEI film is a passivation film formed on the surface of the negative electrode of a lithium-ion battery. It has ionic conductivity and prevents electrons from passing through, separating the electrolyte from the negative electrode. Its formation is a side reaction of the battery at the anode/electrolyte interface, which will lead to irreversible capacity loss and affect the battery’s rate, life and safety.
The SEI film is mainly composed of inorganic and organic substances. For batteries, its thickness can reach more than 100nm. The formation of the SEI film is related to the reaction of lithium ions with the surface of the negative electrode during the charge and discharge process.
Uncontrolled SEI film formation can lead to loss of active materials, reduced battery capacity and increased impedance. SEI film formation under high temperature and high SOC conditions is one of the main causes of battery aging.
Although the SEI film has a certain negative impact on battery performance, a stable SEI film can improve the interface properties of electrode materials and improve battery cycle performance. Optimized formation technology and low-temperature environment help form a dense SEI film, delay the aging process of the battery, and extend the service life.
Current collector corrosion and loss of active material
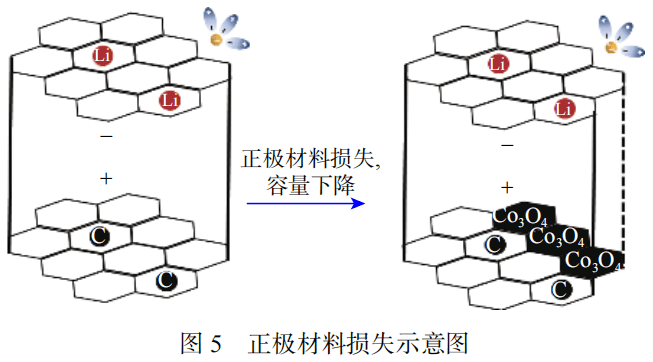
1. Capacity loss caused by current collector corrosion
The current collector is a key component in lithium-ion batteries that carries the active material and collects the output current. Commonly used current collector materials are copper and aluminum. Copper is suitable as a current collector for negative electrode materials, but it is easily oxidized at high potentials.
Aluminum has advantages in terms of cost, mechanical strength, electrical and thermal conductivity, etc., and is generally considered to be one of the most suitable materials as a battery cathode current collector.
Corrosion of the current collector will reduce the life and stability of the battery. Copper current collectors are easily oxidized during excessive discharge, leading to dissolution and increased internal resistance. Aluminum current collectors suffer relatively little corrosion, but there is still room for improvement in stability.
Moisture can inhibit corrosion of aluminum current collectors, but excess moisture can accelerate corrosion. In addition, the thickness change of the copper current collector and the increase of the porous layer will also lead to an increase in the internal resistance.
2. Capacity decline caused by loss of electrode active material
During the charging and discharging process of lithium-ion batteries, the electrode material will undergo volume changes, resulting in mechanical stress. The removal of lithium from the negative electrode material causes volume shrinkage, and the insertion of lithium into the positive electrode material causes volume expansion.
When the volume shrinkage of the negative electrode is greater than the volume expansion of the positive electrode, the overall battery shows volume shrinkage, and vice versa. When charging at a high rate, the battery continues to expand. When charging at a low rate, it expands in the early stages of charging, shrinks in the middle, and expands again in the later stages.
The volume change of the electrode material will generate stress and may cause damage to the negative electrode material. The volume change of the graphite anode during charge and discharge does not exceed 10%, but stress may still cause damage.
The cathode material will also deform. For example, the volume change of lithium iron phosphate material during charge and discharge is about 6.81%, and LiMn2O4 and Mn2O4 are about 6.5%.
Compared with negative electrode materials, positive electrode materials are more susceptible to stress. The diffusion process will increase the lithium ion concentration gradient, causing local volume expansion and generating diffusion-induced stress (DIS). When diffusion-induced stress exceeds a certain threshold, the cathode material particles may break, causing damage. As shown in Figure 5, this phenomenon is more obvious during rapid charge and discharge.
Battery thermal stress is mainly caused by internal temperature differences and temperature changes. Some studies have indirectly characterized the impact of temperature changes on internal stress through changes in battery thickness, but have not conducted a detailed analysis of battery damage caused by thermal stress.
Other studies use simulation modeling methods to quantitatively analyze the influencing factors of thermal stress based on the distribution information of the temperature field and thermal stress field inside the battery.
These studies found that temperatures are highest at the geometric center of the cell, causing this area to expand at high temperatures and be squeezed by stress, while side areas are affected by tensile stresses.
At the same time, concentrated thermal stress may occur at the center of the sides. There are also some studies based on the diffusion-induced stress caused by the difference in lithium ion concentration in the electrode material and the thermal stress generated by the electrochemical cycle, analyzing the impact of volume changes and temperature changes on internal stress during the charge and discharge process.
These studies believe that stress is related to parameters such as charge and discharge rate and battery stack size. In addition, some studies have pointed out that using electrodes made of materials with negative thermal expansion coefficients can effectively eliminate the severe expansion and contraction problems caused by the insertion and extraction of lithium ions.
Electrolyte and separator decomposition
1. The impact of capacity decline caused by electrolyte decomposition
As shown in Figures 6 and 7, the decomposition of the electrolyte will cause the internal resistance of the battery to increase. As the number of cycles increases, the electrolyte will undergo a certain oxidation or decomposition reaction over time, causing its transmission Qualitative ability is weakened.
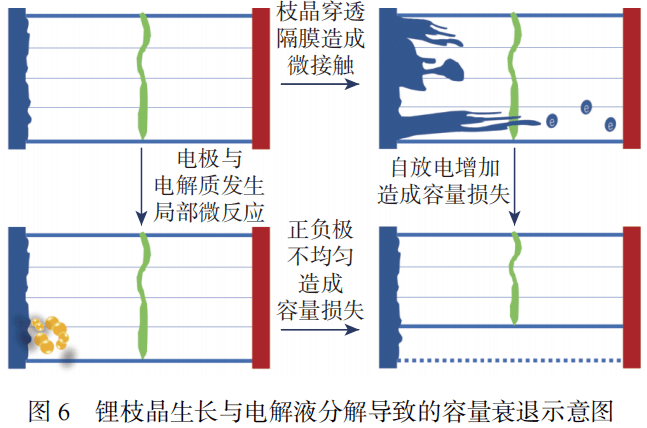
In addition to reacting with the positive and negative electrode surfaces of the battery. When heated, the electrolyte may decompose and produce gases such as CO2, and further increases in temperature may cause the risk of combustion and explosion.
Studies have found that when the operating voltage exceeds the electrochemical stability window of the electrolyte, the electrolyte will undergo an oxidative decomposition reaction with the cathode material. The electrolyte also forms a solid electrolyte interface (SEI) film with the negative electrode and reacts during the lithium evolution process.
In addition, the organic solvent in the electrolyte may undergo transesterification and polymerization reactions, and conductive salts such as LiPF6 will degrade to form organic phosphates and fluorates.
Research also shows that as lithium-ion batteries age, different types of electrolytes will suffer varying degrees of loss, and the LiPF6 concentration will decrease significantly.
2. The impact of separator decomposition on capacity decline
The separator is a key material in lithium-ion batteries, used to separate the positive and negative electrodes and ensure the safe operation of the battery. Excellent separators should have high chemical stability, wettability, thermal stability and mechanical strength, as well as high porosity.
The aging of the separator is mainly due to the clogging of pores, resulting in hindered ion transmission, power attenuation and impedance increase. Causes of separator aging include electrolyte erosion, lithium dendrites passing through the separator pores and structural degradation, while unevenly deposited electrolyte decomposition products can also reduce the ionic conductivity of the separator.
Solutions include asymmetrically modifying the separator surface to inhibit lithium dendrite growth and improve separator life.
Temperature + charge and discharge rate + overcharge
1. Temperature
Ambient temperature has a significant impact on the performance, safety and life of lithium-ion batteries. The suitable operating temperature range is generally 15 to 35 degrees Celsius. At low temperatures, the electrochemical reaction rate slows down, the conductivity of the electrolyte decreases, and the resistance of the solid electrolyte interface (SEI) film increases, resulting in obstruction of lithium ion transmission. Lithium dendrites are easily generated during the charging process, causing irreversible decline in battery capacity and safety risks. .
When working at high temperatures, the electrochemical reaction rate increases, the internal resistance decreases, and the capacity increases. However, sustained high temperatures will accelerate the oxidation and decomposition of the electrolyte, leading to irreversible loss of capacity and increased impedance.
2. Charge and discharge rate
The current rate also affects the capacity and life of lithium batteries. Increasing the charge and discharge rate will accelerate capacity fading and internal resistance growth. Under high-rate charging and discharging, small-capacity batteries are prone to overcharge and over-discharge, accelerating capacity attenuation and forming a positive feedback effect, resulting in reduced available capacity and thermal safety issues.
High-rate charging and discharging will also accelerate battery aging, mainly due to the loss of positive active materials. Battery aging includes two stages: loss of active lithium ions and loss of electrode active materials. Appropriate control of current rate can extend battery life.
3. Analysis of the impact of overcharging on capacity decline
The reasons for battery capacity decline caused by overcharging include lithium precipitation due to overcharging of the negative electrode, gas production due to overcharging of the positive electrode, and aggravation of side reactions when the electrolyte is overcharged. Lithium evolution will reduce the amount of recyclable lithium and trigger side reactions, produce by-products and block the separator pores.
Overcharging of the positive electrode will destroy the capacity balance between the electrodes, lead to irreversible capacity loss, and bring safety risks. Overcharge of the electrolyte will produce by-products and gases, which will clog the electrode pores, increase internal resistance, and form a passivation film to reduce the output voltage.
Battery Difference + Charging Method + Charge and Discharge Deep Cycle
1. Internal inconsistency of the battery
Battery inconsistency is caused by differences in manufacturing processes and usage environments, which will cause the battery pack to accelerate aging under vehicle conditions, affecting the durability, reliability and safety of electric vehicles.
Inconsistency is mainly manifested in parameters such as voltage, internal resistance and capacity, which affects the balance of discharge state and charge and discharge depth. The service life of the battery pack is limited by the minimum life of the single battery. Deep discharge and overload charging and discharging will accelerate aging.
Research has found that the inconsistency of the battery pack is closely related to the inconsistency of the individual cells. There are differences in the actual capacity and charge and discharge depth of different cells, which affects the performance and life of the entire battery pack. Temperature gradients can also cause differences in battery pack aging.
2. Charging forms and strategies
The charging cut-off voltage and fast charging strategy of lithium-ion batteries have an impact on capacity fading and aging. Lowering the charge cut-off voltage increases the usable cycle life but reduces the usable capacity. The aging of fast charging to 100% is more obvious than that of fast charging to 80%, and even the aging of normal charging to 100% is more serious.
Pulse charging can improve charging efficiency and shorten charging time, but it will cause battery aging. Under pulse charging conditions, the internal resistance of the battery increases significantly, and the loss of negative active material becomes more serious. Increasing the pulse frequency does not significantly improve charging efficiency.
3. Charge and discharge depth
Deep charging and discharging accelerates the capacity decline of lithium-ion batteries and increases the ohmic internal resistance and polarization internal resistance. Cycling in the high SOC range is prone to aging, which may be related to the lithium evolution problem in the high SOC range. Constant current charging causes a higher aging rate than constant current and constant voltage charging. Extending the shelf time during charging and discharging or using very small current charging can help extend battery life.
Literature reference: Yan Xiaoyu, Zhou Sida, Lu Yu, Zhou Xin’an, Chen Fei, Yang Shichun, Hua Yang, Xu Kai. Lithium-ion battery capacity decline mechanism and influencing factors [J]. Journal of Beihang University, 2023, 49(6): 1402 -1413
Lithium battery thermal runaway mechanism analysis and control methods!
1. Lithium-ion battery thermal runaway process mechanism
Lithium batteries embed lithium ions into carbon (petroleum coke and graphite) to form a negative electrode. The cathode material is commonly LixCoO2, LixNiO2 and LixMnO4 are also used, and the electrolyte is LiPF6+diethylene carbonate (EC)+dimethyl carbonate (DMC). The main inducing factors of thermal runaway include mechanical damage, overcharging, internal short circuit, etc. Under the influence of various factors, the active materials inside the lithium-ion battery undergo a violent exothermic reaction. After the internal temperature of the battery exceeds the controllable range, it eventually leads to thermal runaway. The exothermic chemical reactions that occur inside the lithium-ion battery include the decomposition of the solid electrolyte interface film SEI, the reaction between the negative active material and the electrolyte, the reaction between the negative active material and the binder, the oxidative decomposition reaction of the electrolyte, etc.
During the charge and discharge process of lithium-ion batteries, the ethylene carbonate on the solid phase interface of the electrode active material will react with the negative electrode lithium to form a layer of SEI film on the graphite adhesion surface. This film can directly slow down or even prevent the reaction between the electrolyte and the active materials on both sides of the electrode, significantly reduce the reaction heat release rate, and improve the stability of the positive and negative electrode materials.
As the temperature rises to 90-120°C, the SEI film begins to decompose, and then an exothermic reaction occurs between the electrolyte and the negative active material. Taking ethylene carbonate as an example, the reaction process is as follows: (1) and (2):
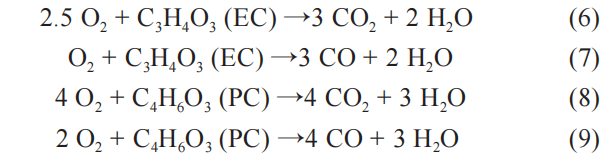
During the exothermic reaction, the internal temperature of the battery gradually increases. Based on the use of different separator materials, their melting points are also different. The melting point of common polypropylene separators is 165°C, and the melting point of polyethylene materials is 135°C. After reaching the melting point temperature of the separator material, the internal separator shrinks locally, causing direct contact between the positive and negative electrode materials inside the battery and causing a short circuit, thus generating a large amount of heat. The large amount of heat generated by the short circuit causes the diaphragm to shrink rapidly, further exacerbating the exothermic reaction.
At the same time, in the temperature range where the SEI film decomposes and undergoes an exothermic reaction, the lithium salt will also undergo a violent exothermic reaction with the electrolyte. Common types of active materials for lithium-ion batteries include lithium hexafluorophosphate (LiPF6), lithium tetrafluoroborate (LiBF4), etc. Lithium hexafluorophosphate decomposes at high temperatures to form PF5. The PF5 produced by the decomposition further reacts with the solvent, absorbs the oxygen atoms of the CO bond, and undergoes a violent exothermic reaction, further accelerating the decomposition of the electrolyte. At the same time, the redox reaction between lithium hexafluorophosphate and the solvent will also release the highly toxic gas hydrofluoric acid (HF). The specific reaction process is as follows: (3)~(5):

Within the same temperature range, the electrolyte itself will undergo a decomposition reaction and release a small amount of flammable gas. When analyzing the thermal runaway process using rate calorimetry, it was found that the gas produced by the decomposition of the electrolyte is mainly composed of C2H4, CO, and H2. The electrolyte is rapidly vaporized and increases the pressure inside the battery. When the internal pressure reaches the limit of the pressure relief valve, a large amount of flammable gas will be ejected, further aggravating the spread of thermal runaway. The heat value generated by the complete combustion of the electrolyte is much greater than the heat released by the decomposition reaction. Taking ethylene carbonate (EC) and propylene carbonate (PC) as examples, the oxidation (6)~(7) and incomplete oxidation of the electrolyte The reaction process of (8)~(9) is as follows:
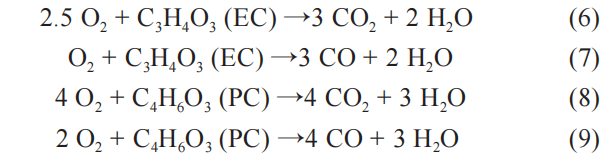
As the internal temperature of the battery gradually increases, the active material of the battery’s positive electrode begins to decompose. Based on the use of different active materials, the temperature at which the exothermic reaction occurs is also different. The positive active material decomposes to produce oxygen, and then the oxygen participates in the reaction with the internal active material, and reacts inside the battery to generate a large amount of gas. The reaction process is as follows:

When the temperature exceeds 136°C, the binder polyvinylidene fluoride (PVDF) will react with lithium to produce hydrogen. The reaction process is as follows:

Except for the SEI film melting and absorbing heat, the above chemical reactions are all exothermic reactions. The heat released by electrolyte decomposition, separators, battery active materials, and adhesives accounted for 43.5%, 30.3%, 20.1%, and 6.2% of the total heat released respectively. The reaction between the positive and negative active materials of the battery and the electrolyte is the largest source of heat release.
2 Factors inducing thermal runaway of lithium-ion batteries
Factors inducing thermal runaway of lithium-ion batteries can be classified into three categories: mechanical abuse (acupuncture, extrusion deformation, external collision), electrical abuse (overcharge, overdischarge, short circuit), thermal abuse (thermal management system failure), etc. Among them, mechanical abuse can easily induce an internal short circuit in the lithium battery, resulting in thermal runaway; during electrical abuse, overcharge and overdischarge of the battery will cause internal side reactions, causing local cells inside the battery to overheat, causing thermal runaway; external short circuit is a kind of battery In the dangerous state of extremely rapid discharge, the extremely high current causes rapid temperature rise and even fuses the battery tabs; in the state of thermal abuse, the thermal management system often fails, inducing the internal diaphragm to shrink and decompose, eventually leading to internal short circuit and thermal runaway.
In addition, the state of the battery itself is also one of the important factors that cause thermal runaway. As the number of battery charge and discharge cycles increases and the impurities mixed in the dendrite production process are induced, adverse side reactions such as metal dendrites are generated that easily pierce the separator. And cause a local short circuit in the battery.
2.1 Research on battery thermal runaway caused by thermal abuse
According to the electrochemical-thermal coupling overcharging-thermal escape model of lithium-ion batteries established in the literature, lithium-ion batteries usually start to self-heat when the temperature reaches 80°C, and battery thermal management occurs when the battery heat overflows and cannot be effectively released. , will cause the battery temperature to rise uncontrollably, spreading from local single cells to the power battery pack, triggering a series of side reactions and thermal runaway. Figure 1 is a schematic diagram of thermal runaway diffusion and temperature changes.
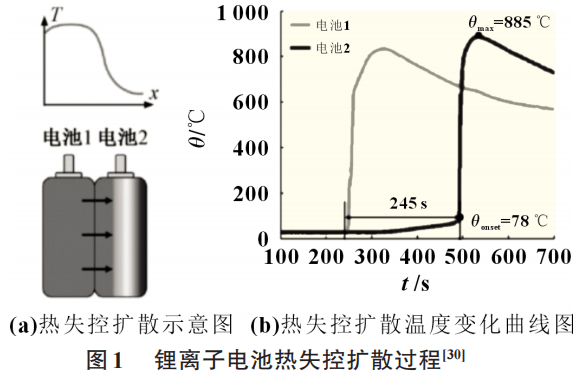
Thermal abuse does not spontaneously occur inside the battery. It is often caused by mechanical abuse and other reasons that cause the internal temperature of the battery to rise to a threshold, and the battery will be heated locally, causing thermal abuse, which further induces temperature control and leads to spontaneous combustion of the battery.
At the same time, thermal runaway is also used as a research method to test the experimental battery runaway process and detect safety characteristics during the battery thermal runaway process. In 1999, KITOH et al. carried out research on monitoring the thermal runaway safety characteristics of high-specific energy power batteries based on external heating methods. Since then, the adiabatic energy method has been widely used to test the thermal runaway temperature threshold of lithium-ion batteries. Current thermal abuse research is mainly based on external radiation igniting batteries. Liu Mengmeng established a multi-endogenous transient heat generation model and an electrochemical-thermal coupling model. Based on the radiation heating method, he studied the safety characteristics of batteries after spontaneous combustion caused by thermal abuse and found that the battery Combustion can be divided into three stages, namely injection combustion, stable combustion and secondary injection combustion. LI et al. studied the effect of discharge current on temperature based on the background of thermal runaway caused by thermal abuse. It was found that when the discharge current is constant, the mass loss, safety characteristic parameters, thermal runaway starting temperature and peak temperature during the thermal runaway process all depend on the battery capacity.
2.2 Research on battery thermal runaway caused by electrical abuse
Common causes of battery thermal runaway include battery overcharge and overdischarge, internal short circuit, external short circuit, etc.
2.2.1 Overcharge and overdischarge
When a lithium-ion battery completes a charge and discharge cycle, the BMS battery management system will block the charging current according to the state of charge under normal circumstances. When the BMS system fails, the battery is overcharged, which can easily cause serious spontaneous combustion accidents. When charging continues after reaching the SOC threshold, lithium metal will adhere to the surface of the negative active material. The attached lithium reacts with the electrolyte at a certain temperature, releasing a large amount of high-temperature gas. At the same time, the positive electrode active material begins to melt due to excessive delithiation and the excessive potential difference with the negative electrode. Once the positive electrode potential is higher than the safe voltage of the electrolyte, the electrolyte will also undergo an oxidation reaction with the positive electrode active material. During the overcharging process, a series of side reactions such as ohmic heating and gas overflow will also occur, exacerbating the occurrence of thermal runaway.
Dr. Ye Jiana found that the gases escaping from lithium-ion batteries during overcharging are mainly composed of CO2, CO, H2, CH4, C2H6 and C2H4, and the gas volume and heat increase as the charging current increases. Using joint analysis with an acceleration calorimeter and a battery cycler, experiments show that overcharging based on constant current-constant voltage is much more dangerous than direct constant current overcharging. Based on the overcharge performance of composite cathodes and graphite anodes in different experimental environments, Ren et al. comprehensively considered the effects of charging current, separator materials, and heat dissipation systems. They found that the amount of heat released by NCM batteries during overcharging is not related to the charging current. Large, different melting points of separator materials and battery deformation and expansion are the main factors causing thermal runaway of lithium-ion batteries. Wang et al. analyzed the heat spread path and high-temperature gas overflow path of overcharged lithium batteries, and found that the heat generated by the reaction between deposited lithium and electrolyte during overcharging of the battery accounted for more than 43%. Zhang et al. studied the degradation mechanism of battery pack capacity based on incremental capacitance-differential voltage and found that a single overcharge has little effect on battery capacity, but after overcharging until the cathode active material is delithiated, it will seriously affect the thermal stability of the battery pack. .
The harm caused by over-discharge is much less. Early over-discharge is difficult to cause thermal runaway of the battery, but it will affect the battery capacity. Zhou Ping et al. studied the discharge characteristics of nickel-cobalt-manganese NCM ternary lithium batteries after overdischarge. During the static discharge process, the degree of short circuit in the NCM lithium battery decreases, the resistance increases, and the discharge current decreases. Experiments show that the greater the depth of discharge, the greater the attenuation of the single cells in the battery pack. Ma et al. found in lithium battery over-discharge experiments that over-discharge will not change the structure of the active material of the battery, but will cause the negative electrode current collector to dissolve, increase the thickness of the SEI film, and accelerate the aging of the battery. The behavior characteristics of lithium-ion battery during over-discharge are shown in Figure 2.
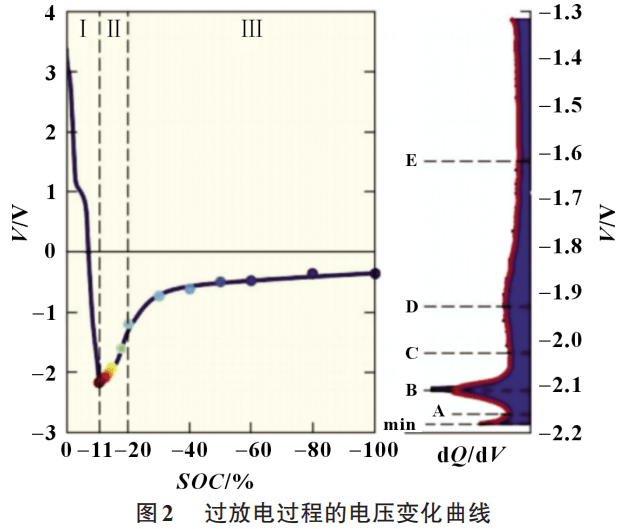
The thermal runaway conditions of different lithium-ion batteries in high and low temperature environments and overcharge and discharge conditions are shown in Figure 3.
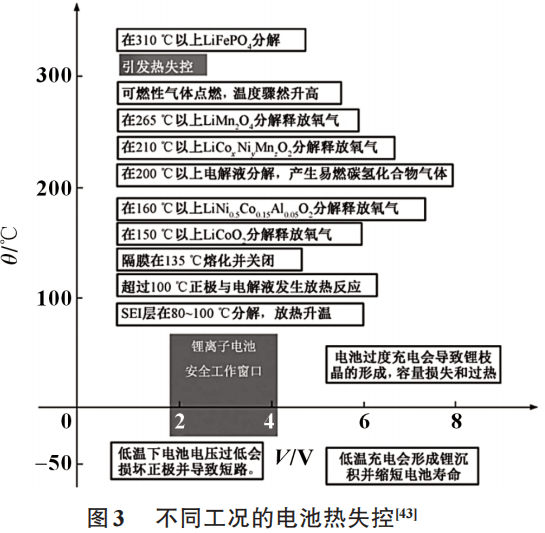
2.2.2 External short circuit
External short circuit is also an important cause of thermal runaway of power batteries. Chen et al. established a new electrothermal coupling model based on the combination of heat generation, distribution, and spread models. Research shows that the peak temperature of a lithium-ion battery exists at the edge of the tab when it is externally short-circuited. Ma Ruxiao et al. found that the heat generated due to side reactions in the external short-circuit state of the power battery is much smaller than the electrochemical heat production, and the electrochemical heat production is positively correlated with the initial SOC, but negatively correlated with the temperature peak thermal stress.
2.2.3 Internal short circuit
Because internal short circuit occurs inside the battery, it is difficult for the BMS system to detect it, which is the main cause of thermal runaway of lithium-ion batteries. When the battery is overcharged or overdischarged, lithium dendrites gradually grow to penetrate the SEI film, causing an internal short circuit and quickly leading to uncontrollable heating and thermal runaway. In addition, lattice damage or collector burrs caused by rough battery manufacturing processes may also cause internal short circuits.
Huang et al. embedded a low-melting point alloy in the diaphragm and punctured it to cause an internal short circuit. They used K-type micro thermocouples to measure the local temperature and collect the heat spread distribution caused by the internal short circuit. Zhang et al. embedded a low deformation temperature threshold nickel-titanium alloy into a separator or current collector and heated it until it deformed and pierced the separator to achieve an internal short circuit. The experiment found that the main source of heat for thermal runaway occurs in the reaction between the positive electrode current collector and the negative electrode, and the short circuit immediately causes a severe temperature rise; while the internal short circuit between the positive electrode and the negative electrode does not cause serious thermal runaway except for partial burning.
2.2.4 Research on battery thermal runaway caused by mechanical abuse
Automotive power batteries inevitably cause mechanical failures due to accidents in applications. If the battery pack is deformed by external forces such as puncture and extrusion, it will cause internal structural changes and even direct contact between the positive and negative electrodes under the stress limit state will cause an internal short circuit and cause thermal runaway. Therefore, it is necessary to study battery thermal runaway based on mechanical abuse. Fan Wenjie and Xu Huiyong et al. have conducted research on thermal runaway caused by mechanical abuse based on finite element modeling and numerical monitoring analysis.
WANG et al. conducted a study based on the change state of the cross-section of the battery pack after a collision of a soft-pack lithium-ion battery. The puncture experiment found that a large number of local deformations and shear fracture layers occurred in the battery pack during the puncture process. The tearing of the current collector and positive active material and the rearrangement of the internal structure of the battery pack caused the separator puncture to be the fundamental cause of short-circuit thermal runaway in the battery. . Lamb et al. studied the deformation state of 18650 cylindrical lithium-ion battery under puncture conditions based on computed tomography technology. The experiment found that the penetration phenomenon between the positive and negative electrodes aggravated the occurrence of internal short circuit. During the short circuit, the attached aluminum foil melted, forming a large number of metal beads at the puncture crack. Li et al. established a finite element analysis model of mechanical abuse in various states based on puncture, extrusion, etc., and used the parameters of used batteries to establish a learning algorithm to predict the process of battery thermal runaway. The impact of mechanical abuse on the safety of lithium-ion batteries is analyzed from eight parameters including impact force, collision angle, and deformation range, which greatly reduces the amount of calculations.
Mechanical abuse that occurs in actual applications is more complex than a single puncture, extrusion and other experiments. It is impossible to deeply study the safety characteristics of mechanical abuse of batteries by relying solely on experimental simulations. The fundamental solution is to optimize the battery installation position while designing the power battery pack. , set up a reliable BMS system and optimize the design of the vehicle frame to minimize the deformation and extrusion of the power battery pack in the event of a collision.
3 Preventive measures and methods for thermal runaway of lithium-ion batteries
With the goal of blocking, delaying, and preventing thermal runaway of power batteries, many scholars have conducted research based on battery pack thermal management and high-strength battery pack structural design.
3.1 Single battery safety design
3.1.1 Research on safety of diaphragm design
The core of improving the safety of the separator is to increase the temperature at which the separator shrinks, melts and decomposes, and enhances the isolation capability under high-temperature conditions. The high-temperature isolation capability of the separator ensures that the micropores of the separator are sealed in high-temperature environments, blocking the outflow of lithium ions. The widely used separator materials are generally covered with ceramic coatings or other materials with closed cell effect.
3.1.2 Research on safety of cathode materials
The most common lithium-ion cathode active materials used in power battery market applications are generally LiCoO2, LiFePO4, LiMn2O4, LiNixCoyMnzO2 (NCM), etc. The positive electrode is covered with materials to block and alleviate thermal runaway side reactions and improve battery cycleability and thermal stability, such as ZrO2 and AlF3. Zhang et al. developed a layered ternary NCM material with atomic concentration based on gradient distribution. Its attached particles are composed of Ni as the core and Mn as the outer layer. Tests show that it can still maintain good cycle performance and thermal stability under multiple high temperatures and overcharge conditions.
3.1.3 Research on safety of negative electrode materials
The improvement of negative electrode safety is mainly through material coating or adding additives to the electrolyte to improve the thermal stability of the SEI film. Xu et al. added liquid alloy GaSnIn to the electrolyte to improve the thermal stability of the battery. Experiments show that the prepared gradient SEI layer greatly reduces voltage polarization and improves Coulomb efficiency to 99.06%. Zheng et al. prepared an ultrathin aramid nanofiber (ANF) film to inhibit lithium dendrite growth. In experimental tests, in a high current density environment of 50mA/cm2, the capacity of the ANF-Li|LiFePO4 full battery decreased to 80.2% after 1,200 cycles. And its research discovered fibrous lithium deposition for the first time. The nanoscale gaps in the prepared ANF film promoted the diffusion of electrolyte, accelerated the efficiency of lithium transport, and eliminated the disadvantages of micron-scale lithium dendrites penetrating the separator.
3.1.4 Research on electrolyte safety
Electrolyte is involved in most thermal runaway accidents, so it is critical to improve electrolyte safety and prevent thermal runaway. Overcharge prevention additives such as flame retardants, solid polymeric substances or ionic liquids are often added to the electrolyte. Fluorinated ethylene carbonate (FEC) is the most common electrolyte additive. Its advantage is to improve the Coulombic efficiency of reversible delithiation of the negative electrode by changing the composition of the SEI membrane. Li et al. used lithium difluoroborate (LiDFOB) as the main salt and designed an SEI membrane between double-layer crystallization and polymer solid electrolyte in a phosphate ester mixed electrolyte. Flame retardant experiments show that the self-extinguishing time of the flame retardant electrolyte is 6.1 seconds, the reversible efficiency of Li is 98.2%, and the battery capacity is still maintained at 89.7% after 150 charge and discharge cycles.
3.2 Power battery system safety protection and optimized design
3.2.1 Battery pack structure optimization design
Battery pack structural design and vehicle installation location optimization are crucial to improving safety. Chen et al. conducted a classification experiment on the impact of thermal runaway range based on the 18650 model battery arrangement. Experiments show that the area with a larger heating area takes a shorter time to ignite, and the spread speed and scope are larger. However, its experiment only considered the overall heating of the power battery module and did not consider the local overheating caused by internal short circuit. Liu Zhenjun and others optimized the battery pack design based on the three-dimensional heat dissipation model of the power battery pack and conducted heat dissipation simulation. Experiments show that the optimized lithium-ion battery peak temperature dropped from 46°C to 34°C, and the temperature difference between single cells was controlled within 5°C.
3.2.2 Battery thermal management system design
Lithium-ion batteries are highly thermally sensitive. Improving low-temperature discharge efficiency and high-temperature safety are the core of the battery thermal management system. Battery pack cooling methods include liquid cooling and air cooling. The electric vehicles produced by Tesla all use liquid cooling technology, and electric buses generally use air cooling. In recent research, aerogels, phase change materials and hybrid materials have been used in battery thermal management systems due to their excellent heat absorption performance. Wu et al. developed a flexible material based on hydrogel for battery thermal management systems. They used low-cost sodium polyacrylate material. Its extremely strong plasticity can be made into various shapes and stacked in battery packs, which can economically realize the traditional The heat dissipation effect of air cooling and liquid cooling.
3.2.3 Cooling, fire extinguishing, blocking and gas guidance design for battery thermal runaway
When battery thermal runaway is unavoidable, it is particularly important to promptly block heat spread, cool down and guide high-temperature gases so as not to affect batteries that are installed in similar locations. Figure 4 is a three-dimensional model of thermal runaway diffusion.
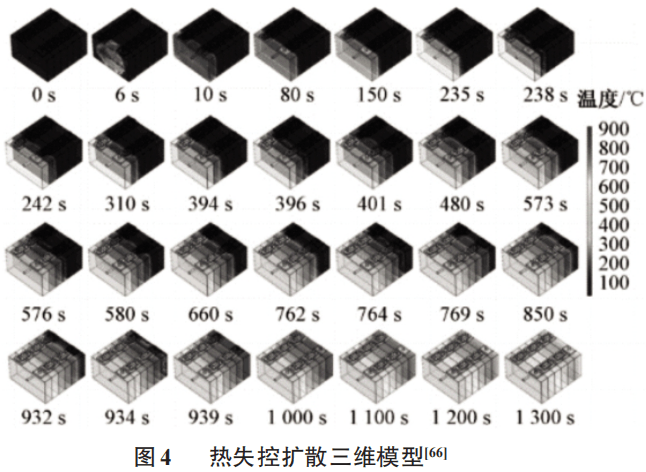
Ways to block the spread of thermal runaway mainly include: filling with flame-retardant media, using insulation materials to isolate the thermal runaway battery, or guiding flames and high-temperature gases through paths to discharge the battery pack. Xu et al. developed a high-temperature gas heat dissipation tube arranged along the battery and with a rectangular cross-sectional shape as shown in Figure 5. Although it cannot prevent the occurrence of thermal runaway of a single battery, it can effectively prevent the spread of local thermal runaway of the battery pack. Li Haoliang et al. designed a heat spread blocking system and integrated control system based on inert gas and mixed refrigerant. The threshold is set for the blocking system based on the heat dispersion map and heating acceleration. Experiments show that the heat spread can be effectively blocked when the battery pack is locally overheated.
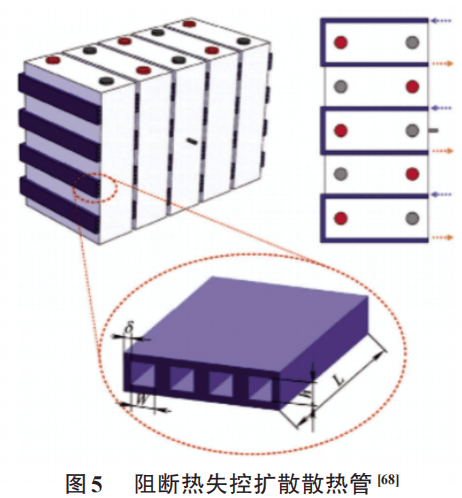
4 Conclusion
This article reviews the literature related to the triggering mechanism, incentives and safety monitoring and management of thermal runaway of lithium-ion power batteries.
(1) In terms of thermal runaway mechanism research, the thermal stability and heat release rules of the main components of lithium-ion batteries are analyzed, and the principles of the reaction heat release processes such as electrolyte decomposition, separators, battery active materials, and adhesives are mainly explained.
(2) In terms of research on thermal runaway trigger triggers, the characteristics and causes of different trigger conditions are classified and summarized, including battery thermal runaway caused by mechanical abuse, electrical abuse and thermal abuse.
(3) In terms of thermal runaway prevention and monitoring, the research on improving the thermal runaway safety of lithium-ion power batteries is elaborated from three aspects: lithium-ion battery cell optimization design, power battery system optimization, and battery thermal management and monitoring and early warning systems.
Although the research on thermal runaway of lithium-ion batteries has achieved a lot of results, there are still gaps in some areas of research. Research on the impact of aging on the safety of lithium-ion batteries due to superposition of cycle times has only begun in recent years. In particular, there are still few experiments on the aging path and mechanism on thermal stability. At the same time, there are only a few experiments on the prediction and modeling of flame spread after thermal runaway occurs, and there is still a lack of numerical simulation analysis of flame propagation. It can be seen that the thermal runaway safety management of lithium-ion power batteries is still in the development stage, especially in the direction of early warning and blocking, which requires more in-depth research.